Thank you for visiting nature.com. You are using a browser version with limited support for CSS. To obtain the best experience, we recommend you use a more up to date browser (or turn off compatibility mode in Internet Explorer). In the meantime, to ensure continued support, we are displaying the site without styles and JavaScript.
Carousel with three slides shown at a time. Use the Previous and Next buttons to navigate three slides at a time, or the slide dot buttons at the end to jump three slides at a time. Pvp K90 Supplier

Junhua Wang, Yun Zhao, … Yushan Yan
Meiling Wu, Xin Zhang, … Liangbing Hu
Lingyou Zeng, Zhonglong Zhao, … Shaojun Guo
Laurie A. King, McKenzie A. Hubert, … Thomas F. Jaramillo
Gautam Das, Bang Ju Park, … Hyon Hee Yoon
Xiao Zhang, Xunhua Zhao, … Haotian Wang
Aaron Hollas, Xiaoliang Wei, … Wei Wang
Junmin Ge, Ling Fan, … Bingan Lu
Fei-Yue Gao, Si-Nan Liu, … Shu-Hong Yu
Scientific Reports volume 5, Article number: 11668 (2015 ) Cite this article
Hydroxide (OH−)-exchange membranes (HEMs) are important polymer electrolytes enabling the use of affordable and earth-abundant electrocatalysts for electrochemical energy-conversion devices such as HEM fuel cells, HEM electrolyzers and HEM solar hydrogen generators. Many HEM cations exist, featuring desirable properties, but new cations are still needed to increase chemical stability at elevated temperatures. Here we introduce the permethyl cobaltocenium [(C5Me5)2Co(III)+ or Cp*2Co+] as an ultra-stable organic cation for polymer HEMs. Compared with the parent cobaltocenium [(C5H5)2Co(III)+ or Cp2Co+], Cp*2Co+ has substantially higher stability and basicity. With polysulfone as an example, we demonstrated the feasibility of covalently linking Cp*2Co+ cation to polymer backbone and prepared Cp*2Co+-functionalized membranes as well. The new cation may be useful in designing more durable HEM electrochemical devices.
Polymer hydroxide (OH−)-exchange membranes (HEMs) are attractive electrolytes for electrochemical energy conversion devices such as fuel cells1,2,3,4,5,6,7,8,9,10,11,12,13,14,15,16,17,18, electrolyzers19,20 and solar hydrogen generators21, largely due to their intrinsic compatibility with non-precious-metal catalysts2,22 and superior CO2 tolerance23 compared to liquid alkaline electrolytes24. The active hydroxide-conducting component of an HEM is a cation that is covalently linked to a polymer backbone. Organic cations based on nitrogen [ammonium25,26, pyridinium27, guanidinium28,29, imidazolium6,30], phosphorus [phosphonium3,8], sulfur [sulfonium31] and ruthenium [bis(terpyridine)ruthenium7] have been introduced, featuring specific HEM properties including improved solubility3, enhanced thermal stability31 and increased basicity32. New cations with chemical stability at elevated temperatures (>80 °C33,34) are still desired to reduce CO2 poisoning, increase catalyst activity and improve heat management in HEM electrochemical devices.
Alkali metal cations (e.g., Li+, Na+ and K+) provide the highest OH− conductivity and show excellent stability, but at present cannot be covalently tethered to a polymer backbone for HEM applications35. Organic bis(cyclopentadienyl) metallocenium cations based on VIIIB family metals [(C5H5)2M(III)+ or Cp2M(III)+, M = Co, Rh, Ir, or Mt] satisfy the 18-valence electron stability rule and resemble alkali metal cations: They bear one unit of positive charge, may be precipitated by the addition of excess anions and with hydroxide as counter-ion absorb CO2 and water from ambient air36. In particular, cobalt is the smallest atom in the VIIIB family and forms the strongest metal-ring bonds, resulting in the most stable metallocenium cation, Cp2Co+ (cobaltocenium)37. While Cp2Co+ has been used in strongly basic anion-exchange resins38,39 and water-soluble redox-active oligomers/polymers40,41, none of these compounds appears suitable for HEM applications. In addition, the stability of Cp2Co+ may be still limited due to its unsubstituted Cp rings.
Here we introduce the concept of using permethyl cobaltocenium [(C5Me5)2Co(III)+ or Cp*2Co+, Fig. 1a,b] as a highly stable cation for polymer HEMs that are required in designing able and durable electrochemical devices.
Structure of Cp*2Co+ cation and Cp*2Co+-functionalized polysulfone (Cp*2Co+-PSf).
(a) Chemical structure of Cp*2Co+. (b) Molecular structure of Cp*2Co+. (c) Chemical structure of Cp*2Co+-PSf. (d) Molecular structure of Cp*2Co+-PSf (one repeat unit of polysulfone shown, predicted by the software MOPAC and drawn in Jmol, version 13.0).
Complete methylation of the Cp ring in Cp*2Co+ enhances electron donation to the metal center, strengthening the metal-ring bond and delocalizing positive charge away from the metal center42. Calculations confirm that Cp*2Co+ has a lower heat of formation (ΔHf) than Cp2Co+, suggesting stronger bonding: 499 vs. 775 kJ mol−1 (a 36% reduction), as predicted by the semi-empirical quantum chemistry software MOPAC2012 (Table 1). The same calculation confirms that the charge on cobalt (δCo) is lower in Cp*2Co+ than in Cp2Co+: +0.988 vs. +1.058 e (a 6.6% reduction). (Note that the overall system charge remains exactly +1 for both cations, regardless of delocalization.) This reduced charge is consistent with the substantial negative shift (ca. 600 mV) in redox potential (φ) observed for Cp*2Co(III)+/Cp*2Co(II) vs. Cp2Co(III)+/Cp2Co(II) (−1.24 V vs. −0.63 V, referring to the standard hydrogen electrode, SHE, with CH2Cl2 as solvent43).
The reduced positive charge on the metal center makes Cp*2Co+ less susceptible to nucleophilic attack by hydroxide, which is, in general, the first step in HEM degradation. In addition to the electronic effect, steric hindrance42 from the methyl groups in the Cp* ligands may also further protect Cp*2Co+ from hydroxide attack. The difference in steric hindrance between Cp*2Co+ and Cp2Co+ can be quantified6 by comparing the accessible angle (θ) that is formed by the cobalt center and the edges of circumcircle of hydrogen atoms (Fig. S1). Cp2Co+ has an accessible angle of 72.4° while Cp*2Co+ has much smaller accessible angles of 40.3–49.6° (Table 1). The smaller accessible angle reduces the chance for the cobalt atom to be attacked.
Indeed, a high-temperature alkaline stability test (at 140 °C in 1 M NaOD/D2O) showed that Cp*2Co+ is significantly more stable than Cp2Co+. After six weeks (1,000 hours) only 8.5% of the initial Cp*2Co+ had been found to degrade (13C NMR, Fig. S2) whereas Cp2Co+ degraded completely after only one week (Fig. S3). Further, Cp*2Co+ showed no change in UV-vis absorption after the stability test (Fig. S4). For comparison, a typical ammonium cation (trimethyl benzylammonium) degraded by 18% in 24 hours (Fig. S5). Cp*2Co+ demonstrates a level of stability that has not been achieved by any known HEM cations (Fig. 2).
Alkaline stability of Cp*2Co+ and other reported cations.
Test conditions: 1 M KOD or NaOD in D2O, 20% degradation threshold on 1H NMR basis (unless otherwise noted). Cobaltocenium: Cp*2Co+ (13C NMR spectroscopy, this work). Ammonium: benzyl-trimethylammonium (btmAm) [80 °C in 1 M NaOD/(D2O+CD3OD)8 and 100 °C in 1 M NaOD/D2O, this work]. Imidazolium: benzyl-1-methyl-limidazolium (bmIm)67, 1,3-dimethyl-2-phenyl-benzimidazolium (dmpBIm) (0.3 M KOH)6, 1,3-dimethyl-2-(2,4,6-trimethylphenyl)-benzimidazolium (dmtmpBIm) (1.3 M KOH)6. Guanidinium: benzyl-pentamethylguanidium (bpmGu) (this work). Pyridinium: benzylpyridinium (bPy) (this work). Phosphonium: tetrakis(dialkylamino)phosphonium (tkdaaPh) [1 M NaOD/(D2O+CD3OD)]8. Sulfonium: (4-methoxyphenyl)-diphenylsulfonium (mopdpSu)31. Ruthenium: bis(terpyridinine)ruthenium (ttpRu) (UV-vis spectroscopy). The chemical structures of those cations are shown in Table S3. 1H NMR spectra of btmAm, bpmGu and bPy are not shown.
The reduced charge on cobalt also improves the basicity of the cation hydroxide, since weakened cation-anion interaction favors dissociation. Indeed, the base dissociation constant (Kb) is much larger for Cp*2Co+OH− than for Cp2Co+OH−: pKb = 2.7 vs. 5.4 (a 500-fold increase in Kb, Table 1), as measured by correlating OH− concentration with total organic base concentration in aqueous solution (Table S1 and Fig. S6). Improved basicity is expected to increase the hydroxide conductivity of the corresponding HEMs.
Superior stability and basicity suggest that Cp*2Co+ is a much more desirable cation than Cp2Co+ for HEM applications.
Cp*2Co+-PSf was synthesized by a diamine-bridge strategy (Fig. S7). The feasibility of this approach was established by a series of small-molecule model reactions (bromination, Fig. S8; anion exchange, Fig. S9; amination, Fig. S10; and cation incorporation, Fig. S11), in which benzyl chloride functioned as a surrogate for the halomethylated polymer. The Cp*2Co+ cation was first brominated to introduce a single halomethyl group. In addition to 1H NMR spectroscopy (Fig. S8), the bromination step was further confirmed by 13C NMR (Fig. S12) and mass spectroscopy (Fig. S13, no sign of multiple bromination). In parallel, one amine group from hexamethylenediamine (HMDA) was linked to benzyl chloride by amination (leaving the other amine group intact). Then, the remaining unreacted amine group from HMDA was used to link the brominated Cp*2Co+. Reaction conditions for all steps were optimized (yield >95%, 1H NMR spectroscopy).
In a similar manner, Cp*2Co+-PSf was synthesized (Figs. S14 and S15) and then membranes were prepared. Unlike the small-molecule model reaction, proper reaction conditions are critical for amination of polysulfone. Firstly, a low reaction temperature (e.g., 20 °C) avoids the ammonolysis that leads to polysulfone depolymerization (observed at 40 °C and higher). Secondly, a large excess of amine (e.g., 20 equiv.) avoids cross-linking in which one amine molecule reacts with two chloromethyl groups. Once cross-linked, the polymer becomes insoluble and is no longer suitable for further reactions.
Note that the hydrophobic44,45 PF6− anion in Br-Cp*2Co+PF6− must be exchanged for a hydrophilic anion (e.g., Cl−) prior to linking the cation to the backbone, due to the difficulty of exchanging PF6− for the ultimately desired OH− in polyelectrolytes7. The counter-ion was later exchanged from Cl− to OH− with 1 M KOH. The Cp*2Co+-PSf membranes were found be to flexible, uniform in thickness and transparent (Fig. 3a).
Characterization of Cp*2Co+-PSf membrane.
(a) Photograph (2” x 2”, 100 μm thick). (b) Dynamic mechanical analysis (DMA) test curve (ambient humidity and temperature, 10 mm min−1 cross-head speed). (c) Thermal gravimetric analysis (TGA) and derivative thermal gravimetric (DTG) curves (10 °C min−1, nitrogen atmosphere).
Due to the presence of cobalt, high-contrast transmission electron microscopy (TEM) images were obtained without the need to employ a dye anion (e.g., PdCl42− 46 or WO42− 16), directly revealing detailed features of the membrane microstructure (Fig. S16a). Overall, the hydrophilic (dark) and hydrophobic (light) domains are homogenously interspersed, which is essential to simultaneously provide ionic conduction and mechanical robustness16. The hydrophilic domains show an average size of 15 nm, similar to those in typical ammonium-based membranes (10–30 nm) with the same polysulfone backbone and similar ion exchange capacity (IEC, 1.14 mmol/g)46. The presence of cobalt was also confirmed by energy dispersive X-ray (EDX) spectroscopy during imaging (Fig. S16b). High tensile strength (40 MPa with 10% elongation at break, Fig. 3b) shows that the membrane is strong and robust. Under similar test conditions, the commercial proton exchange membrane (PEM) Nafion® 212 is half as strong (around 20 MPa47).
Two membranes of different degrees of functionalization (DFs, 100% and 123%) were characterized (Table 2). Both hydroxide conductivity and water uptake increase with ion exchange capacity (IEC), as with HEMs based on other cations. Note that the measured IECs are very close to the theoretical ones: 1.04 vs. 1.09 mmol g−1 for Cp*2Co+-PSf100 membrane; 1.16 vs. 1.20 mmol g−1 for Cp*2Co+-PSf123 membrane. Such an agreement indicates the Cp*2Co+ cations in Cp*2Co+-PSf membranes are fully functional. For comparison, HEMs with similar theoretical IEC and similar backbone (when available) but different cations are summarized in Table 2. The Cp*2Co+-PSf membranes are similar to other membranes in terms of hydroxide conductivity (10–22 vs. 0.19–45 mS cm−1) and water uptake (41%–68% vs. 8.2%–240%) in deionized water at room temperature, as well as IEC-normalized hydroxide conductivity (HCIEC, 9.2–18 vs. 0.15–38 mS∙g cm−1 mmol−1), suggesting similar cation basicity and hydroxide conduction efficiency. As expected, hydroxide conductivity of Cp*2Co+-PSf membranes increases with temperature. E.g., the hydroxide conductivity of Cp*2Co+-PSf123 membranes reached 49 and 64 mS cm−1 at 60 and 80 °C, respectively.
The membranes showed very high thermal stability, consistent with the aforementioned stability of the Cp*2Co+ cation. The onset of decomposition temperature of 305 °C (Fig. 3c) is the highest among all reported HEMs: e.g., 60 °C higher than a sulfonium-functionalized PSf31, 120 °C higher than a phosphonium-functionalized PSf48, or 150 °C higher than ammonium-functionalized PSfs46,49. Note that the thermal gravimetric analysis (TGA) data only reflect short-term thermal stability and are only useful for comparison under similar test conditions.
The Cp*2Co+-PSf membranes also showed improved alkaline stability: At 80 °C in 1 M KOH, the IEC loss of Cp*2Co+-PSf membranes was 18% and 27% of the initial value after 1,000 and 2,000 hours, respectively (Fig. S17). After the 1,000-hour stability test, solid-state 13C NMR spectroscopy confirmed that Cp*2Co+ functional groups remained almost unchanged (Fig. S18), but there were clear signs of PSf backbone scission. This result is consistent with that of a recent study50 and it further highlights the need for more stable backbones in developing next-generation highly durable HEMs.
Under a more aggressive test at 100 °C for 2,000 hours, the IEC lost was about 50% for Cp*2Co+-PSf membranes. Considering that higher test temperature leads to shorter membrane lifetime, the membrane lifetime is plotted against the test temperature (Fig. S19). With 20% loss of initial IEC as the failure criterion, Cp*2Co+-PSf membranes are more stable than all other cation-based HEMs reported to date.
Polysulfone was used as the polymer backbone in this work primarily to demonstrate linking Cp*2Co+ to polymer and to compare Cp*2Co+ to other cations reported. However, polysulfone backbone is sensitive for HEM degradation50,51 and more stable polymer backbones are needed to better match the stable Cp*2Co+ cation. Very recently, alternative polymer backbones, such as polystyrene52 and poly(phenylene)53, have been shown to have better stability than polysulfone in alkaline media. In principle, the synthesis reported here may be modified to employ those more stable polymer backbones. The incorporation of Cp*2Co+ cation to more stable polymer backbones and the synthesis and stability of their resulting membranes warrant important future research.
In the amination step, the chain length of the diamine linker between the polymer backbone and the Cp*2Co+ cation was found to affect membrane flexibility, likely resulting from the incompatibility between the rigid hydrophobic polymer backbone and the bulky hydrophilic cation. Increasing the chain length (e.g., ethylene54, propylene54,55 and hexamethylene56,57,58) between cation and backbone helps alleviate this incompatibility. Indeed, HMDA, with six carbon atoms and two nitrogen atoms, was found to be a good choice for preparing flexible, robust membranes.
In the cation incorporation step, although Br-Cp*2Co+PF6− could react at two possible amine sites — either close to the polymer backbone (the basal secondary amine) and or at the end of side chain (the terminal primary amine) (Fig. S7) — a reaction was only observed at the terminal site, even for the less-hindered model molecule (Fig. S10). Such good selectivity results from strong steric hindrance in the bulky Br-Cp*2Co+PF6− molecule and higher nucleophilicity of the terminal amine group (pKa of amines’ conjugate acids: 10.21 and 10.21 for the basal amine vs. 9.26 and 9.93 for the terminal amine, of HMDA-aminated chloromethylated polysulfone polymer and HMDA-aminated benzyl chloride small molecule, respectively; calculated by the MarvinSketch software, Table S2).
During the model cation durability test, 13C instead of 1H NMR spectroscopy was used to monitor stability because both Cp*2Co+ and Cp2Co+ undergo rapid H-D isotopic exchange upon exposure to alkaline media, rendering 1H spectroscopy inappropriate for accessing stability52. Figs. S20 and S21 show the degree of H-D isotopic exchange is 78% and 29% for Cp*2Co+ and Cp2Co+, respectively, under the same test conditions (60 °C, 40% KOH/D2O in methanol, 30 min). This difference in degree of exchange is consistent with the observation from the literature that methyl protons are slightly more acidic than ring protons in cobaltocenium [pKa in methanol: 22.7 for methyl protons of 1,1’-dimethyl-cobaltocenium, (1-Me-Cp)2Co+, vs. 23.9 for ring protons of Cp2Co+]59,60.
Table 2 summarizes the HEM samples with similar backbone and IECs for the purpose of comparing different cations. It is noted that HEMs with very high hydroxide conductivity have been reported. In general, high conductivity can be achieved by providing either high IEC or high efficiency of hydroxide conduction. With high IECs (> 2.0 mmol g−1), HEMs exhibited high hydroxide conductivities (at ~20 °C unless otherwise noted) such as 43, 50, 65, 67 and 68.7 mS cm−1 for a badmAm-based PPO (2.75 mmol g−1)9, a btmAm-based fluorenyl-containing poly(ether sulfone ketone) (2.54 mmol g−1, 30 °C)61, a btmAm-based di-fluorinated bpPSf (2.62 mmol g−1)62, a bpmGu-based bpPSf (2.15 mmol g−1)28 and a badmAm-based cross-linked polyalkylene (2.3 mmol g−1)5, respectively. High conductivities can also be realized with relatively low IECs via methods that likely improve the efficiency of hydroxide conduction. Examples include the use of architecture of aliphatic side chain (45 mS cm−1, 30 °C, with 1.0 mmol g−1 for a btmAm-based hexyl-modified PSf)13, saturated aliphatic backbone (48 mS cm−1 with 1.5 mmol g−1 for a trimethyl ammonium-based polyalkylene)63, all-benzene-ring backbone (50 mS cm−1 with 1.57 mmol g−1 for a btmAm-based poly(phenylene)64, hydrogen-bonding group (1,2,3-triazole) (62 mS cm−1 with 1.8 mmol g−1 for a trimethyl ammonium-based triazole-containing PPO)65 and block copolymer backbone (80 mS cm−1 with 1.93 mmol g−1 for a poly(fluorenyl sulfone)-co-PSf)16.
In summary, Cp*2Co+ shows improved stability and basicity compared with Cp2Co+, making Cp*2Co+ an ultra-stable cation for polymer HEMs. We have also incorporated Cp*2Co+ cations to a typical polymer backbone (polysulfone) and the resulting membranes exhibited enhanced (short-term) thermal stability and improved (long-term) alkaline stability. More stable polymer backbones are needed to better match the outstanding stability of Cp*2Co+ cation; and the availability of such a stable organic cation might help to develop the next-generation affordable and durable HEM electrochemical devices.
Heat of formation in gas phase (ΔHf) of the cation and partial charge at the cobalt atom (δCo) were calculated by the software MOPAC2012 (Stewart Computational Chemistry) through the graphical user interface Vega ZZ (version 3.0.1.22, Drug Design Laboratory at the University of Milan). The keyword CHARGE =1 was used to specify the total charge of the cation and the keyword PRECISE was used to tighten convergence criteria. Conjugate acid dissociation constants (Ka) of two amine groups (basal and terminal) in hexamethylenediamine connected to polysulfone polymer and a benzyl group were predicted using the pKa module of the software MarvinSketch (version 5.12.3, ChemAxon Ltd.) through an empirically parameterized method based on partial charge distribution66. One repeat unit of polysulfone with hydrogen atoms as end-groups was used in place of the whole polymer for the pKa calculation.
Consider the dissociation equilibrium of a general base: MOH M+ + OH−. In the equilibrium state, Kb = ([M+]∙[OH−])/[MOH], where Kb is the dissociation constant of the base; [M+], [OH−] and [MOH] are concentrations of dissociated M+, dissociated OH− and undissociated MOH, respectively. When [H+] is neglected (reasonable in base), [M+] = [OH−], so Kb = [OH−]/(C0/[OH−]−1), where C0 is the initial concentration of MOH. Kb can be obtained from fitting the slope of the line of [OH−] vs. (C0/[OH−]−1). The pH of Cp*2Co+OH− and Cp2Co+OH− solutions was measured at different initial concentrations (ranging from 0.25 to 50 mM) and the slope was fitted to give Kb. Pure Cp*2Co+OH− and Cp2Co+OH− were prepared by ion exchange of Cp*2Co+PF6− and Cp2Co+PF6−, respectively, with a strongly-basic fast-kinetics anion-exchange resin (Amberjet® 4200, Dow Chemical Co.), which had been pre-exchanged with hydroxide in 50 wt% water : 50 wt% methanol, followed by filtration to remove resin and evaporation to remove solvent.
Permethyl-cobaltocenium hexafluorophosphate (Cp*2Co+PF6−) was brominated by N-bromosuccinimide (NBS) with dibenzoyl peroxide (BPO) as radical initiator and 1,1,2,2-tetrachloroethane (TCE) as solvent. Specifically, 0.474 g (1 mmol) of Cp*2Co+PF6− was dissolved in 10 ml TCE and then 0.178 g (1 mmol) of NBS and 0.0121 g (0.05 mmol) of BPO were added into the solution. After 24 hours of reaction with stirring at 100 °C, brominated permethyl-cobaltocenium hexafluorophosphate (Br-Cp*2Co+PF6−) was precipitated by pouring the reacted solution into excess diethyl ether. Subsequently, the Br-Cp*2Co+PF6− precipitate was filtered, washed thoroughly with diethyl ether and dried in vacuum at room temperature. The degree of bromination was found to be 95% by NMR spectroscopy (1H, Fig. S8 and 13C, Fig. S12) and mass spectroscopy (Fig. S13). Second, the Br-Cp*2Co+PF6− was ion exchanged with a strongly basic fast-kinetics anion exchange resin balanced with Cl− to produce brominated permethyl-cobaltocenium chloride (Br-Cp*2Co+Cl−). Specifically, Br-Cp*2Co+PF6− was dissolved in deionized water and then the excess (20 equiv.) anion exchange resin (Amberjet® 4200 with chloride anion) was added into the solution. With stirring, the ion exchange was held at room temperature for 24 hours and then the resin was removed by filtration. Br-Cp*2Co+Cl− was obtained as a yellow powder by evaporating water from the filtrate. The anion exchange was confirmed by 19F NMR spectroscopy with 97% replacement of PF6− by Cl− (Fig. S9).
First, benzyl chloride was aminated by excess (20 equiv.) hexamethylenediamine (HMDA). Specifically, 0.127 g (1 mmol) benzyl chloride was dissolved in 10 ml N-methyl-2-pyrrolidone (NMP), then 2.32 g (20 mmol) HMDA and 0.652 g (2 mmol) cesium carbonate were added into the solution. The amination reaction was carried out for 24 hours with stirring at room temperature, then the solvent was removed by adding excess diethyl ether, leaving behind the mixture of desired product (i.e., N-benzylhexane-1,6-diamine, BHDA) and residual HMDA. The HMDA was removed by addition of excess saturated K2CO3 aqueous solution. The leftover BHDA white power was thoroughly washed with deionized water, followed by drying at 40 °C for 48 hours under vacuum. The synthesis was confirmed by 1H NMR spectroscopy with complete mono-amination (Fig. S10). Second, (6-(benzyl amino)hexylamino permethyl-cobaltocenium chloride (BAHA-Cp*2Co+Cl−) was synthesized by reacting BHDA with Br-Cp*2Co+Cl−. Specifically, 0.206 g (1 mmol) BHDA was dissolved in 10 ml NMP and 0.444 g (1 mmol) Br-Cp*2Co+Cl− was added into the solution. The reaction was carried out at 80 °C for 24 hours with stirring, then the solvent was removed by adding excess diethyl ether and the BAHA-Cp*2Co+Cl− was obtained after washing by saturated K2CO3 aqueous solution and deionized water sequentially. The reaction was confirmed by 1H NMR spectroscopy with complete conversion (Fig. S11) and complete selectivity for the terminal amine over the basal amine.
First, chloromethylated polysulfone (CM-PSf) was synthesized with trimethylchlorosilane and paraformaldehyde as co-chloromethylating agent and stannic chloride as catalyst as reported in detail in our previous work32. Second, CM-PSf was aminated with excess HMDA similar to the case of benzyl chloride. Specifically, 0.502 g (1 mmol polysulfone repeat unit or 1.23 mmol chloromethyl group for 123% of DC) CM-PSf was dissolved in 10 ml NMP, then 2.85 g (24.6 mmol) HMDA and 0.802 g (2.46 mmol) cesium carbonate were added into the solution. The amination reaction was held at room temperature for 24 hours with stirring, then the HMDA-aminated polysulfone (HMDA-PSf) white powder was precipitated by pouring the reacted mixture into excess deionized water. After filtration and thorough washing with deionized water, the HMDA-PSf was dried at 40 °C for 48 hours under vacuum. The synthesized HMDA-PSf is completely soluble in organic solvents such as chloroform and NMP and neither crosslinking nor depolymerization was found for the HMDA-PSf. The synthesis was confirmed by 1H NMR spectroscopy with 96% conversion of the chloromethyl groups (Fig. S14).
Second, Cp*2Co+-PSf was synthesized by reacting HMDA-PSf with Br-Cp*2Co+Cl−. Specifically, 0.600 g (1 mmol polysulfone repeat unit or 1.23 mmol terminal amine group) HMDA-PSf was dissolved in 10 ml NMP, then 0.546 g (1.23 mmol) Br-Cp*2Co+Cl− was added into the solution. The reaction was completed by heating the mixture at 80 °C for 24 hours with stirring. The reacted solution was cast onto a glass plate and the Cp*2Co+-PSf membranes were obtained after drying at 60 °C for 72 hours. The balancing anion of Cp*2Co+-PSf membranes was exchanged with hydroxide by treatment in 1 M KOH at room temperature for 48 hours. After thorough washing and immersion in deionized water for another 48 hours to completely remove residual KOH, the membranes were ready for use. The synthesis of Cp*2Co+-PSf was confirmed by solid-state 13C NMR spectroscopy (Fig. S15).
How to cite this article: Gu, S. et al. Permethyl Cobaltocenium (Cp*2Co+) as an Ultra-Stable Cation for Polymer Hydroxide Exchange Membranes. Sci. Rep. 5, 11668; doi: 10.1038/srep11668 (2015).
Varcoe, J. R. & Slade, R. C. T. Prospects for alkaline anion-exchange membranes in low temperature fuel cells. Fuel Cells 5, 187–200 (2005).
Lu, S. F. et al. Alkaline polymer electrolyte fuel cells completely free from noble metal catalysts. P Natl Acad Sci Usa 105, 20611–20614 (2008).
Gu, S. et al. A Soluble and Highly Conductive Ionomer for High-Performance Hydroxide Exchange Membrane Fuel Cells. Angew Chem Int Edit 48, 6499–6502 (2009).
Clark, T. J. et al. A Ring-Opening Metathesis Polymerization Route to Alkaline Anion Exchange Membranes: Development of Hydroxide-Conducting Thin Films from an Ammonium-Functionalized Monomer. J Am Chem Soc 131, 12888–12889 (2009).
Article CAS PubMed Google Scholar
Robertson, N. J. et al. Tunable High Performance Cross-Linked Alkaline Anion Exchange Membranes for Fuel Cell Applications. J Am Chem Soc 132, 3400–3404 (2010).
Article CAS PubMed Google Scholar
Thomas, O. D. et al. A Stable Hydroxide-Conducting Polymer. J Am Chem Soc 134, 10753–10756 (2012).
Article CAS PubMed Google Scholar
Zha, Y. P. et al. Metal-Cation-Based Anion Exchange Membranes. J Am Chem Soc 134, 4493–4496 (2012).
Article CAS PubMed Google Scholar
Noonan, K. J. T. et al. Phosphonium-Functionalized Polyethylene: A New Class of Base-Stable Alkaline Anion Exchange Membranes. J Am Chem Soc 134, 18161–18164 (2012).
Article CAS PubMed Google Scholar
Li, N. W., Leng, Y. J., Hickner, M. A. & Wang, C. Y. Highly Stable, Anion Conductive, Comb-shaped Copolymers for Alkaline Fuel Cells. J Am Chem Soc 135, 10124–10133 (2013).
Article CAS PubMed Google Scholar
Zeng, R. et al. Alkaline ionomer with tuneable water uptakes for electrochemical energy technologies. Energy Environ Sci 4, 4925–4928 (2011).
Deavin, O. I. et al. Anion-exchange membranes for alkaline polymer electrolyte fuel cells: comparison of pendent benzyltrimethylammonium- and benzylmethylimidazolium-head-groups. Energy Environ Sci 5, 8584–8597 (2012).
Pan, J. et al. A strategy for disentangling the conductivity-stability dilemma in alkaline polymer electrolytes. Energy Environ Sci 6, 2912–2915 (2013).
Pan, J. et al. Constructing ionic highway in alkaline polymer electrolytes. Energy Environ Sci 7, 354–360 (2014).
Li, N. W. et al. Comb-shaped polymers to enhance hydroxide transport in anion exchange membranes. Energy Environ Sci 5, 7888–7892 (2012).
John, J. et al. An Electrochemical Quartz Crystal Microbalance Study of a Prospective Alkaline Anion Exchange Membrane Material for Fuel Cells: Anion Exchange Dynamics and Membrane Swelling. J Am Chem Soc 136, 5309–5322 (2014).
Article CAS PubMed Google Scholar
Tanaka, M. et al. Anion Conductive Block Poly(arylene ether)s: Synthesis, Properties and Application in Alkaline Fuel Cells. J Am Chem Soc 133, 10646–10654 (2011).
Article CAS PubMed Google Scholar
Varcoe, J. R. et al. Anion-exchange membranes in electrochemical energy systems. Energ Environ Sci 7, 3135–3191 (2014).
Wang, Y. et al. Pt-Ru catalyzed hydrogen oxidation in alkaline media: oxophilic effect or electronic effect? Energy Environ Sci 8, 177–181 (2015).
Xiao, L. et al. First implementation of alkaline polymer electrolyte water electrolysis working only with pure water. Energy Environ Sci 5, 7869–7871 (2012).
Leng, Y. J. et al. Solid-State Water Electrolysis with an Alkaline Membrane. J Am Chem Soc 134, 9054–9057 (2012).
Article CAS PubMed Google Scholar
Spurgeon, J. M. et al. Electrical conductivity, ionic conductivity, optical absorption and gas separation properties of ionically conductive polymer membranes embedded with Si microwire arrays. Energy Environ Sci 4, 1772–1780 (2011).
Gu, S. et al. An efficient Ag-ionomer interface for hydroxide exchange membrane fuel cells. Chem Commun 49, 131–133 (2013).
Adams, L. A. et al. A carbon dioxide tolerant aqueous-electrolyte-free anion-exchange membrane alkaline fuel cell. Chemsuschem 1, 79–81 (2008).
Article CAS PubMed Google Scholar
Gu, S., Xu, B. J. & Yan, Y. S. Electrochemical Energy Engineering: A New Frontier of Chemical Engineering Innovation . Annu Rev Chem Biomol Eng 5, 429–454 (2014).
Article CAS PubMed Google Scholar
Agel, E., Bouet, J. & Fauvarque, J. F. Characterization and use of anionic membranes for alkaline fuel cells. J Power Sources 101, 267–274 (2001).
Danks, T. N., Slade, R. C. T. & Varcoe, J. R. Comparison of PVDF- and FEP-based radiation-grafted alkaline anion-exchange membranes for use in low temperature portable DMFCs. J Mater Chem 12, 3371–3373 (2002).
Huang, A. B., Xiao, C. B. & Zhuang, L. Synthesis and characterization of quaternized poly(4-vinylpyridine-co-styrene) membranes. J Appl Polym Sci 96, 2146–2153 (2005).
Wang, J. H., Li, S. H. & Zhang, S. B. Novel Hydroxide-Conducting Polyelectrolyte Composed of an Poly(arylene ether sulfone) Containing Pendant Quaternary Guanidinium Groups for Alkaline Fuel Cell Applications. Macromolecules 43, 3890–3896 (2010).
Article ADS CAS Google Scholar
Kim, D. S., Labouriau, A., Guiver, M. D. & Kim, Y. S. Guanidinium-Functionalized Anion Exchange Polymer Electrolytes via Activated Fluorophenyl-Amine Reaction. Chem Mater 23, 3795–3797 (2011).
Guo, M. L. et al. Synthesis and characterization of novel anion exchange membranes based on imidazolium-type ionic liquid for alkaline fuel cells. J Membrane Sci 362, 97–104 (2010).
Zhang, B. Z. et al. Tertiary sulfonium as a cationic functional group for hydroxide exchange membranes. RSC Adv 2, 12683–12685 (2012).
Gu, S. et al. Quaternary Phosphonium-Based Polymers as Hydroxide Exchange Membranes. Chemsuschem 3, 555–558 (2010).
Article CAS PubMed Google Scholar
Pivovar, B. 2011 Alkaline Membrane Fuel Cell Workshop Final Report. National Renewable Energy Laboratory, Arlington, Virginia, (2011) Date of Access: 01/01/2013.
Sturgeon, M. R. et al. Hydroxide based Benzyltrimethylammonium Degradation: Quantification of Rates and Degradation Technique Development. J Electrochem Soc 162, F366–F372 (2015).
Luo, H. Z. et al. Anion exchange membrane based on alkali doped poly(2,5-benzimidazole) for fuel cell. Solid State Ionics 208, 52–55 (2012).
Wilkinson, G. & Cotton, F. A. Cyclopentadienyl and Arene Metal Compounds. Prog Inorg Chem 1, 1–124 (1959).
Sheats, J. E., Hlatky, G. & Dickson, R. S. Stabilization of Negative Charge by the Cobalticinium Nucleus .3. Effect of Substituents on the Acidities of Hydroxy-Cobalticinium and Hydroxy-Rhodicinium Salts. J Organomet Chem 173, 107–115 (1979).
Ito, T. & Kenjo, T. Ion-Exchange Properties of Anion-Exchanger Containing Cobalticinium Cations. B Chem Soc Jpn 41, 1600-& (1968).
Ito , T. & Kenjo , T. A New Anion Exchanger Containing Cobalticinium Cation .B Chem Soc Jpn 41, 614-& (1968).
Mayer, U. F. J., Gilroy, J. B., O’Hare, D. & Manners, I. Ring-Opening Polymerization of 19-Electron [2]Cobaltocenophanes: A Route to High-Molecular-Weight, Water-Soluble Polycobaltocenium Polyelectrolytes. J Am Chem Soc 131, 10382-+ (2009).
Article CAS PubMed Google Scholar
Ren, L. X., Hardy, C. G. & Tang, C. B. Synthesis and Solution Self-Assembly of Side-Chain Cobaltocenium-Containing Block Copolymers. J Am Chem Soc 132, 8874-+ (2010).
Article CAS PubMed Google Scholar
Robbins, J. L., Edelstein, N., Spencer, B. & Smart, J. C. Syntheses and Electronic-Structures of Decamethylmetallocenes. J Am Chem Soc 104, 1882–1893 (1982).
Koelle, U. & Khouzami, F. Permethylated Electron-Excess Metallocenes. Angew Chem Int Edit 19, 640–641 (1980).
O’Mahony, A. M. et al. Effect of Water on the Electrochemical Window and Potential Limits of Room-Temperature Ionic Liquids. J Chem Eng Data 53, 2884–2891 (2008).
Terborg, L. et al. Ion chromatographic determination of hydrolysis products of hexafluorophosphate salts in aqueous solution. Anal Chim Acta 714, 121–126 (2012).
Article CAS PubMed Google Scholar
Pan, J. et al. High-Performance Alkaline Polymer Electrolyte for Fuel Cell Applications. Adv Funct Mater 20, 312–319 (2010).
Dai, H. et al. Properties and fuel cell performance of proton exchange membranes prepared from disulfonated poly(sulfide sulfone). J Power Sources 185, 19–25 (2008).
Gu, S., Cai, R. & Yan, Y. S. Self-crosslinking for dimensionally stable and solvent-resistant quaternary phosphonium based hydroxide exchange membranes. Chem Commun 47, 2856–2858 (2011).
Cao, Y. C., Wang, X., Mamlouk, M. & Scott, K. Preparation of alkaline anion exchange polymer membrane from methylated melamine grafted poly(vinylbenzyl chloride) and its fuel cell performance. J Mater Chem 21, 12910–12916 (2011).
Arges, C. G. & Ramani, V. Two-dimensional NMR spectroscopy reveals cation-triggered backbone degradation in polysulfone-based anion exchange membranes. P Natl Acad Sci Usa 110, 2490–2495 (2013).
Patel, N. B. & Patel, J. C. Synthesis and antimicrobial activity of Schiff bases and 2-azetidinones derived from quinazolin-4(3H)-one. Arab J Chem 4, 403–411 (2011).
Nunez, S. A. & Hickner, M. A. Quantitative H-1 NMR Analysis of Chemical Stabilities in Anion-Exchange Membranes. Acs Macro Letters 2, 49–52 (2013).
Choe, Y. K. et al. Alkaline Stability of Benzyl Trimethyl Ammonium Functionalized Polyaromatics: A Computational and Experimental Study. Chem Mater 26, 5675–5682 (2014).
Zhang, Q. A. et al. Synthesis and alkaline stability of novel cardo poly(aryl ether sulfone)s with pendent quaternary ammonium aliphatic side chains for anion exchange membranes. Polymer 51, 5407–5416 (2010).
Zhang, Q. A., Li, S. H. & Zhang, S. B. A novel guanidinium grafted poly(aryl ether sulfone) for high-performance hydroxide exchange membranes. Chem Commun 46, 7495–7497 (2010).
Lin, B. C. et al. A Soluble and Conductive Polyfluorene Ionomer with Pendant Imidazolium Groups for Alkaline Fuel Cell Applications. Macromolecules 44, 9642–9649 (2011).
Article ADS CAS Google Scholar
Qiu, B. et al. Bis-imidazolium-based anion-exchange membranes for alkaline fuel cells. J Power Sources 217, 329–335 (2012).
Hibbs, M. R. Alkaline Stability of Poly(phenylene)-Based Anion Exchange Membranes With Various Cations. J Polym Sci Pol Phys 51, 1736–1742 (2013).
Bykova, E. V., Mukhina, T. A., Setkina, V. N. & Kursanov, D. N. Effect of Substituents on Rate of Protophilic Isotopic-Exchange of Hydrogen of Cobalticinium Salts. Doklady Akademii Nauk Sssr 214, 329–331 (1974).
Sheats, J. E., Miller, W. & Kirsch, T. Stabilization of Negative Charge by Cobalticinium Nucleus. J Organomet Chem 91, 97–104 (1975).
Tanaka, M., Koike, M., Miyatake, K. & Watanabe, M. Anion Conductive Aromatic Ionomers Containing Fluorenyl Groups. Macromolecules 43, 2657–2659 (2010).
Article ADS CAS Google Scholar
Wang, J. H. et al. Synthesis of Soluble Poly(arylene ether sulfone) Ionomers with Pendant Quaternary Ammonium Groups for Anion Exchange Membranes. Macromolecules 42, 8711–8717 (2009).
Article ADS CAS Google Scholar
Kostalik, H. A. et al. Solvent Processable Tetraalkylammonium-Functionalized Polyethylene for Use as an Alkaline Anion Exchange Membrane. Macromolecules 43, 7147–7150 (2010).
Article ADS CAS Google Scholar
Hibbs, M. R., Fujimoto, C. H. & Cornelius, C. J. Synthesis and Characterization of Poly(phenylene)-Based Anion Exchange Membranes for Alkaline Fuel Cells. Macromolecules 42, 8316–8321 (2009).
Article ADS CAS Google Scholar
Li, N. W., Guiver, M. D. & Binder, W. H. Towards High Conductivity in Anion-Exchange Membranes for Alkaline Fuel Cells. Chemsuschem 6, 1376–1383 (2013).
Article CAS PubMed Google Scholar
Szegezdi, J. & Csizmadia, F. in American Chemical Society Spring Meeting (Chicago, IL, 2007).
Wang, J. H. et al. Stabilizing Imidazolium Cation for Hydroxide Exchange Membranes. ChemSusChem 6, 2079–2082 (2013).
Article CAS PubMed Google Scholar
Opitz, J. Electron impact ionization of cobalt-tricarbonyl-nitrosyl, cyclopentadienyl-cobalt-dicarbonyl and biscyclopentadienyl-cobalt: appearance energies, bond energies and enthalpies of formation. Int J Mass Spectrom 225, 115–126 (2003).
Zarrin, H., Wu, J., Fowler, M. & Chen, Z. W. High durable PEK-based anion exchange membrane for elevated temperature alkaline fuel cells. J Membrane Sci 394, 193–201 (2012).
Li, N. et al. Phenyltrimethylammonium Functionalized Polysulfone Anion Exchange Membranes. Macromolecules 45, 2411–2419 (2012).
Article ADS CAS Google Scholar
Zhang, Y. M. et al. Novel fluoropolymer anion exchange membranes for alkaline direct methanol fuel cells. J Colloid Interf Sci 381, 59–66 (2012).
Article ADS CAS Google Scholar
Pan, J., Li, Y., Zhuang, L. & Lu, J. T. Self-crosslinked alkaline polymer electrolyte exceptionally stable at 90 degrees C. Chem Commun 46, 8597–8599 (2010).
Wang, X. et al. A polytetrafluoroethylene-quaternary 1,4-diazabicyclo-[2.2.2]-octane polysulfone composite membrane for alkaline anion exchange membrane fuel cells. Int J Hydrogen Energ 36, 10022–10026 (2011).
Arges, C. G. et al. Assessing the influence of different cation chemistries on ionic conductivity and alkaline stability of anion exchange membranes. J Mater Chem 22, 3733–3744 (2012).
Valade, D., Boschet, F., Roualdes, S. & Ameduri, B. Preparation of Solid Alkaline Fuel Cell Binders Based on Fluorinated Poly(diallyldimethylammonium chloride)s [Poly(DADMAC)] or Poly(chlorotrifluoroethylene-co-DADMAC) Copolymers. J Polym Sci Pol Chem 47, 2043–2058 (2009).
Xia, Z. J. et al. Polybenzimidazoles with pendant quaternary ammonium groups as potential anion exchange membranes for fuel cells. J Membrane Sci 390, 152–159 (2012).
Ni, J. et al. Novel self-crosslinked poly(aryl ether sulfone) for high alkaline stable and fuel resistant alkaline anion exchange membranes. Chem Commun 47, 8943–8945 (2011).
Yan, X. M. et al. Imidazolium-functionalized polysulfone hydroxide exchange membranes for potential applications in alkaline membrane direct alcohol fuel cells. Int J Hydrogen Energ 37, 5216–5224 (2012).
Lin, B. C., Qiu, L. H., Lu, J. M. & Yan, F. Cross-Linked Alkaline Ionic Liquid-Based Polymer Electrolytes for Alkaline Fuel Cell Applications. Chem Mater 22, 6718–6725 (2010).
Page, O. M. M. et al. The alkali stability of radiation-grafted anion-exchange membranes containing pendent 1-benzyl-2,3-dimethylimidazolium head-groups. RSC Adv 3, 579–587 (2013).
Wang, J. H. et al. Stabilizing The Imidazolium Cation for Hydroxide Exchange Membranes. Chemsuschem 6, 2079–2082 (2013).
Article CAS PubMed Google Scholar
Henkensmeier, D. et al. Polybenzimidazolium hydroxides - Structure, stability and degradation. Polym Degrad Stabil 97, 264–272 (2012).
Zhang, C. X. et al. Microphase separated hydroxide exchange membrane synthesis by a novel plasma copolymerization approach. J Power Sources 198, 112–116 (2012).
This work was supported by the MURI of the U.S. DOD under the contract W911NF-10-1-0520. S.G. thanks Mr. Jesse McAtee and Dr. Guangjin Hou in the Department of Chemistry and Biochemistry of University of Delaware for the help in mass spectroscopy study and in solid-state NMR spectroscopy investigation, respectively.
Department of Chemical & Biomolecular Engineering, Center for Catalytic Science and Technology, University of Delaware, Newark, 19716, Delaware, USA
Shuang Gu, Junhua Wang, Robert B. Kaspar, Qianrong Fang, Bingzi Zhang & Yushan Yan
Department of Polymer Science and Engineering, University of Massachusetts, Amherst, 01003, Massachusetts, USA
You can also search for this author in PubMed Google Scholar
You can also search for this author in PubMed Google Scholar
You can also search for this author in PubMed Google Scholar
You can also search for this author in PubMed Google Scholar
You can also search for this author in PubMed Google Scholar
You can also search for this author in PubMed Google Scholar
You can also search for this author in PubMed Google Scholar
S.G. designed the experiments. S.G. and J.H.W. performed the polymer synthesis experiments, Kb measurement and membrane characterization. J.H.W. performed the model reaction experiments and TEM imaging. Q.R.F. and J.H.W. performed cation stability test. B.Z.Z. and J.H.W. performed NMR test. R.B.K. performed the computational predictions on partial charge, heat of formation, molecular structure and pKa. E.B.C. designed the studies of benchmark ammonium degradation, mass spectroscopy and H-D isotopic exchange experiments. S.G., E.B.C. and Y.S.Y. wrote the manuscript. Y.S.Y. directed the research work.
The authors declare no competing financial interests.
This work is licensed under a Creative Commons Attribution 4.0 International License. The images or other third party material in this article are included in the article’s Creative Commons license, unless indicated otherwise in the credit line; if the material is not included under the Creative Commons license, users will need to obtain permission from the license holder to reproduce the material. To view a copy of this license, visit http://creativecommons.org/licenses/by/4.0/
Gu, S., Wang, J., Kaspar, R. et al. Permethyl Cobaltocenium (Cp*2Co+) as an Ultra-Stable Cation for Polymer Hydroxide-Exchange Membranes. Sci Rep 5, 11668 (2015). https://doi.org/10.1038/srep11668
DOI: https://doi.org/10.1038/srep11668
Anyone you share the following link with will be able to read this content:
Sorry, a shareable link is not currently available for this article.
Provided by the Springer Nature SharedIt content-sharing initiative
By submitting a comment you agree to abide by our Terms and Community Guidelines. If you find something abusive or that does not comply with our terms or guidelines please flag it as inappropriate.
Scientific Reports (Sci Rep) ISSN 2045-2322 (online)
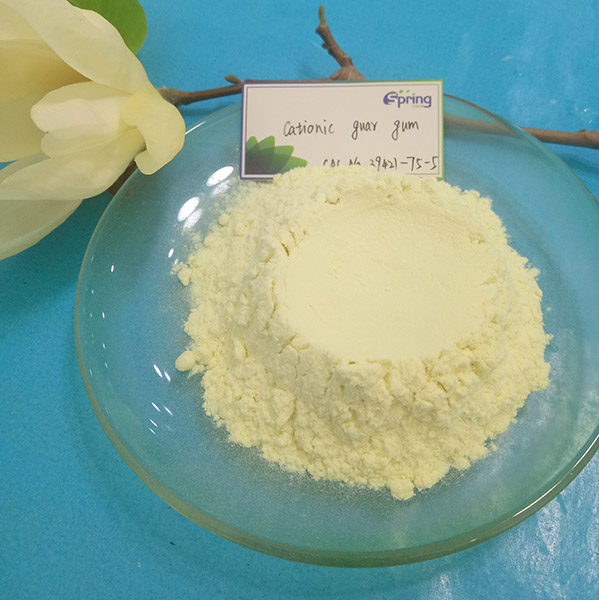
Activated Poly Sodium Metasilicate Sign up for the Nature Briefing newsletter — what matters in science, free to your inbox daily.